Chapter 4: Proteins as Antenna
According to the prevalent mechanistic paradigm, the properties of a given substances, whether a proteins, an element, or a therapeutic molecule is defined by its chemical composition.
However, research shows that two molecules having the very
same composition but exhibiting
different geometry (conformation) display radically different properties:
The vast majority of possible protein molecules could adopt many conformations of roughly equal stability,
each conformation having different chemical properties.
[1]
Actually, the geometric shape of a protein is so important in determining its properties that any alteration of its 3D structure will modify its function:
Protein function is directly related to the structure of that protein. A protein's specific shape determines its function. If the three-dimensional structure of the protein is altered because of a change in the structure of the amino acids, the protein becomes denatured and does not perform its function as expected.
[2]
The above strongly suggest that the properties of a protein - fundamentally its informational content - are not so much defined by its chemical composition but by its geometric shape.
The preeminence of shape over chemical composition is perfectly illustrated by the concept of chirality, also known as handedness.
Chirality refers to two entities being the mirror image of each other. It’s the case of our hands, being the same, except that they are mirror image of each other. The same goes for a pair of shoes for example: the two shoes are the same: same material, same size, same composition except they are the mirror image of each other, in other terms their image cannot be superposed.
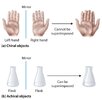
© Libretext
(a) Objects that are non-superimposable mirror images of each other are chiral,
(b) The flask is achiral because it can be superimposed on its mirror image.
Chirality is not restricted to macroscopic objects like shoes, golf clubs or corkscrews; it also applies to small entities like molecules.
An interesting example is Vitamin C, also known as ascorbic acid. Its formula is C6H8O, but it exists as two enantiomers (mirror-image isomers), "L" (for "Levo" or left) and "D" (for "Dextro" or right). Although the L and the D molecule of vitamin C have exactly the same chemical composition; their properties are drastically different
[3], while the L conformation exhibits numerous beneficial healing properties the R isomer is neutral in most cases.
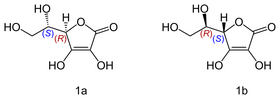
© Yikrazuul
Isomers of vitamin C: L-ascorbic acid (1a); D-ascorbic acid (1b)
Vitamin C is just one example among many others. Two enantiomers of a same compound can significantly differ in their taste, smell or other biological actions.
For example, (+)-limonene found in orange (causing its smell), and (–)-limonene found in lemons (causing its smell), show different smells
[4] . The same applies to carvone: (+)-carvone is responsible for the smell of caraway seed oil, whereas (–)-carvone is responsible for smell of spearmint oil.
[5]
The same goes for pharmaceutical molecules, two enantiomers sometimes show remarkable difference in terms of biological actions
[6]. For instance, D-propoxyphene
[7] is a documented painkiller, whereas its enantiomer, L-propoxyphene
[8] is an effective anti-cough agent.
In the same vein, the S-isomer of
penicillamine, is a treatment of primary chronic arthritis, whereas the R-isomer has no therapeutic effect and is highly toxic.
[9]
The preeminence of geometry over composition in defining the properties - informational content - of a substance was explained, until recently, by the key-locks model invented by German chemist
Emil Fischer in 1894
[10]. According this simple model - chirality matters only on a mechanistic level: the geometric shape of a protein allows or not its binding to a specific receptor. In this context, the “fitting” is mechanical, it occurs between a pattern along the key and the counter pattern within the lock.
But, in today’s technology, remote control keys do not have any pattern fitting at all. The key sends an electronic signal which enables the locking, or the unlocking of the lock.
[11]. Could it be same at the cellular scale?
Fortunately, since the 1890’s, then numerous direct and indirect observations of “binding” sites have revealed that the lock and key analogy was a simplistic mechanistic analogy unable to account for numerous documented features of intercellular dynamics:
in terms of vibrations, the human body can be compared to a symphony orchestra. Each molecule corresponds to a particular instrument. Each bend, rotation, or stretch of a chemical bond has a certain resonant frequency and will give off certain frequencies or 'notes' if it is energized. Since molecules, water, and dissolved ions are constantly bumping into each other at body temperature, all parts are constantly jiggling and absorbing and emitting characteristic frequencies.
While a chemical process, such as the breaking of a bond, may look superficially like a mechanical event, at a deeper level the event is better described as a series of vibratory energetic interactions. This is one level at which the various energy therapies may have their effects.
A soprano shatters a crystal goblet by singing a high note coinciding with the natural frequency of the goblet. The atoms in the glass vibrate so strongly that they cannot hold together, and the gobelet breaks. The same thing can happen to a molecule. Figure 9.10 shows a molecule of hydrogen Peroxide, H2O2, being fractured by vibrations. Sometimes this is called 'molecular surgery'.
The oxygen--oxygen (0-0) bond of hydrogen peroxide is broken selectively with an electromagnetic field of a specific frequency. The wavy arrow in the drawing represents a photon of laser light that energizes the O—H bond (i.e., it makes the bond vibrate violently) just as a tuning fork resonates when you strike it. The vibrations are rapidly conducted or redistributed throughout the molecule, and the 0-0 bond, which is weaker than the 0—H bond, breaks.
[12]
Random diffusion, which is the biochemical mechanism of action fostered by the lock and key model is simply too imprecise (random) and too slow (diffusion) to account for numerous biological processes:
random diffusion is far too slow and far too imprecise to regulate living processes in an orderly timely manner. Diffusion of regulatory molecules and diffusion of metabolites within cells re processes that are too slow to allow the organism to adapt to rapid changes would take about in its environs-
lent' If random diffusion is the mechanism involved in cellular metabolism,1,000 years for you to digest your breakfast! [13]
The lock and key model is a materialist theory based on random mechanical process. In this sense the lock and key model is to biochemistry what Darwinism is to “evolution”.
Random mutation has as little chance to explain evolution - the chance that a tornado sweeping through a junkyard might assemble a Boeing 747, according to Fred Hoyle
[14] - as random diffusion can explain biochemistry.
The lock and key model postulates that the random diffusion of a ligand is what directs its binding of the to its receptor. It’s akin to being blindfolded, holding a key, not knowing where the lock is, poking the key here and there, and hoping the key will magically end up in the right position, the right location, in the right lock at once!
At least in some cases, if not in most cases, the biological communication processes don’t follow the imprecise, slow lock and key model but the fast and precise resonance model:
© Oschman
The lock and key model (A) VS the resonance model (B)
The resonance model is not a novelty; it was created by Malcom Dyson in 1937
[15]. Since then, this theory has been experimentally confirmed in various fields.
The lock and key model repeatedly failed to predict the potency of a given agonist, on the contrary to the resonance model for which both the shared spectral peak of related agonists and the intensity of this peak are predictors of agonist potency
[16]:
Although the lock and key mechanism has been very successful in modelling
certain aspects of the action of signaling proteins one of the ways in which it falls short is the prediction of agonist potency. In addition to the shared spectral peak of related agonists
Hoehn et al. also report that the intensity of this peak might be used as a predictor of agonist potency
[17]
The above suggests that the ability of a protein to interact with a receptor is not its geometric shape but its resonance characteristics.
The resonance model of molecular interactions has been particularly investigated in the domain of olfaction (sense of smell). For example, experiments on fruit flies showed a resonance phenomenon in the neuro-receptors related to smell:
In his 1996 paper
Turin suggested, that the
vibrational frequency of a given odorant contributes to quantum tunneling at the receptor. In 2011
Franco et al. presented experimental evidence in support of the theory. In particular they claimed that fruit flies could differentiate between deuterated odorants.
[18]
A
deuterated odorant refers to a molecule where a usual hydrogen atom (one proton + one electron) was replaced by a deuterium atom (one proton + one neutron + one electron).
© NASA
Hydrogen and deuterium atoms
This minute addition of one neutron to the hydrogen nuclei occurs only at the
atomic level. It doesn’t change at all the odorant at a
molecular level, therefore falsifying the lock and key model, which is based on geometry differences on a
molecular level.
The addition of one neutron in the deuterium atom compared to the hydrogen atoms modifies the geometric shape on an
atomic level and the whole resonance properties of the molecule
[19] constituted by these atoms.
The ability of the fruit flies to smell a difference between deuterated and un-deuterated odorants suggests that the perception of smell is not defined by the composition of the molecule, but the geometric shape at an atomic scale and the subsequent vibrational signature.
In 2001, a study conducted at Purdue University showed that, like fruit flies, humans can also perceive the difference in smell between normal and deuterated versions of a given molecule.
[20]
Similar experiments have shown that lake white fish and the American cockroach can differentiate between
isotopes of the same amino acids and pheromones
[21]. Like in deuterated vs hydrogenated odorants, the difference between isotopes of a same element is only the number of neutrons in the nucleus of the atom.
But, agonist prediction and smell biochemistry are only some of the domains were the resonance model has been successfully tested. Literally thousands of proteins revealed resonance interactions at a distance:
In our previous studies, the above criteria have been tested with
over 1000 proteins from 25 functional groups. The following fundamental conclusion was drawn from our studies:
one RRM [Resonant Recognition Model] peak frequency characterizes one particular biological function or interaction. Therefore, those peaks are named as the RRM characteristic frequencies. It was shown that proteins and their interacting targets (receptors, binding proteins, and inhibitors) display the same characteristic frequency in their interactions. However, it is obvious that one protein can participate in more than one biological process, i.e. revealing more than one biological function. Despite proteins and their targets have different biological functions they can participate in the same biological process. Therefore, it has been postulated that interacting molecules "communicate" with each other, i.e. recognise each other at a distance, on the basis of the same/similar (within the calculation error) characteristic frequency but opposite phases at that frequency
[22]
The cases of resonances in proteins interactions are so prevalent, that after repeatedly analyzing the spectrum of the distribution of the energies of free electrons along proteins backbone,
Emiritus professor
Irena Cosic concluded:
protein activities (interactions) are based on
resonant electromagnetic energy transfer.
[23]
From the above we now know that two substances exhibiting the very same composition reveal drastically different properties depending on their geometric shape, whether on a molecular scale as illustrated by chiral molecules or on an atomic scale as exemplified by the isotope experiments.
These properties of a given substance are defined by its specific connection with the information field. In this case, one can state that the properties of a substance are defined by the specific “area” of the information field it is connected to, this specific connection is defined by the geometric shape of the substance, which modulates its resonance signature.
The above shows that while the mechanistic lock and key model can explain
some biochemical processes, it fails to explain others for which resonance seems to be involved.
So, in the next chapters, we will attempt to understand how the geometric shape of a given substance is associated with its resonance and with its informational content.
Why two molecules displaying the exact same composition differ so much in properties/informational content? We know that geometric shape obviously plays a fundamental role, but how does it work? Is the difference in geometry altering a fundamental factor involved in modulating the connection between the information field and the proteins? What is this fundamental factor?
The next chapter aims at answering these questions.
[1] Alberts, B
. et al. (2002) “Molecular Biology of the Cell. 4th edition”.
Garland Science in The Shape and Structure of Proteins
[2] Smith, Yolanda (2018) “
Protein Structure and Function” News-Medical
[3] Wu X,
et al. (2020) “A chirality-dependent action of vitamin C in suppressing Kirsten rat sarcoma mutant tumor growth by the oxidative combination: Rationale for cancer therapeutics”
Int J Cancer 146(10):2822-2828
[4] Solomon and Fryhles. “Organic chemistry”, Ed 10,
Wiley (Students edition), Chapter 5 (Stereochemistry), Section 5.5 More about biological significance of chirality
[5] Ibid
[6] Sanganyado,
et al. (2017) "Chiral pharmaceuticals: A review on their environmental occurrence and fate processes".
Water Research. 124: 527–542.
[7] brand name
Darvon
[8] brand name
Novrad
[9] Solomon and Fryhles organic chemistry, Ed 10, Wiley (Students edition), Chapter 5 (Stereochemistry), section 5.11(chiral drugs).
[10] Liu, Shu-Qun
et al. (2012) “Protein Folding, Binding and Energy Landscape: A Synthesis” in Protein engineering
Kaumaya
[11] Arieh Ben-Naim (2018) “Solvent Effects and the Lock and Key Model for Molecular Recognition”
Int J Bio & Lab Med. 1:1, 01-06
[12] Eden, Donna & Feinstein, David (1999) “Energy Medicine”
Tarcher/Putnam p.137
[13] Ibid, p.133
[14] Gatherer, Derek (2008) "Finite Universe of Discourse: The Systems Biology of Walter Elsasser (1904-1991)"
The Open Biology Journal. 1: 9–20
[15] Hoehn RD
et al. (2018) “Status of the Vibrational Theory of Olfaction”
Front. Phys. 6:25
[16] An agonist is a chemical that “binds” to a receptor and activates the receptor to produce a biological response. In contrast, an antagonist blocks the action of the agonist.
[17] Hoehn RD
et al. (2015) “Neuroreceptor activation by vibration-assisted tunneling”.
Sci Rep.;5:9990
[18] Adams, B.
et al. (2020) “Quantum effects in the brain: A review”
AVS Quantum Science. 2. 022901 10.1116/1.5135170
[19] More specifically it adds vibrational modes of the carbon-deuterium bond. See:
A. P. Horsfield
et al. (2017). “Molecular recognition in olfaction”
Advances in Physics: X, 2:3, 937-977
[20] Haffenden LJ, Yaylayan VA, Fortin J (2001). ‘’Investigation of vibrational theory of olfaction with variously labelled benzaldehydes”.
Food Chem. 73 (1): 67–72
[21] Havens BR, Melone CD (1995) “The application of deuterated sex pheromone mimics of the American cockroach, to the study of wright's vibrational theory of olfaction”.
Dev. Food. Sci. 37 (1): 497–524
[22] Cosic, I., Pirogova, E. (2007) “Bioactive peptide design using the Resonant Recognition Model”.
Nonlinear Biomed Phys 1, 7
[23] Cosic, Irena. (2001) “The Resonant Recognition Model of Bio-molecular Interactions: possibility of electromagnetic resonance”
Polish Journal of Medical Physics And Engineering. 7. 73-87